Re-growing limbs and organs in order to recover from irrevocable physical losses is no longer just for science fiction characters. Due to the advancements in regenerative medicine and tissue engineering, recovery of this magnitude is now possible. Regenerative medicine is the process of creating functional tissues to replace tissues or organs that have been damaged by disease, trauma, or congenital issues, and create solutions for organs that have become permanently damaged. The terms regenerative medicine and tissue engineering have become interchangeable; however, tissue engineering is a concentration or subfield of regenerative medicine. The focus of tissue engineering is to create constructs that restore, maintain, or improve damaged tissue or whole organs through the process of combining scaffolds, cells, and biologically active molecules into functional tissue [1,2].
The Department of Defense (DoD) supports regenerative medicine in the hope of treating and curing fatal and debihilitating conditions. According to a study completed by the U.S. Government Accountability Office, between 2012 and 2014 the DoD was the second largest funder of regenerative medicine. The DoD invested $253 million into 178 projects related to regenerative medicine and health needs of active-duty military personnel [3].
According to Stratistics Market Research Consulting, the global tissue engineering market is expected to reach $16.82 billion by 2023 [4]. Three different areas categorize the tissue engineering market: synthetic, biological, and genetically engineered solutions. Generally, the synthetic products currently available (e.g., polypropylene hernia meshes) can be manufactured on a large scale, but do not provide the environment required for cells inside the body to properly grow and integrate into the mesh, resulting in severe scar formation. The biological solutions (i.e., allografts) can be integrated into the body, but suffer from problems with variable outcomes such as premature degradation, immune system reactions, sourcing of materials, and high cost [5]. The genetically engineered solutions typically consist of cells grown in a bioreactor in the lab to create a functional organ that can be implanted, but this is hugely expensive and still in early development. ParaGen Technologies aims to redefine tissue engineering through the development of a new type of synthetic scaffold capable of rapid biointegration and highly reproducible patient outcomes. The synthetic scaffold also ensures no immune rejection and high function regrowth. The core technology is a nanofiber scaffold that mimics physical structures (see Figure 1) found within the body that allows for assimilation and tissue level structural support. The nanofiber scaffold is made from completely synthetic polymers (e.g., the same polymers used in resorbable sutures such
as polyglycolide) and resorbs over time so no foreign material remains in the body.
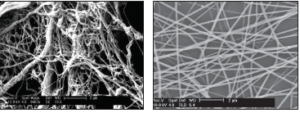
Figure 1. (Left) Scanning electron microscope image of a decellurized blood vessel demonstrating the fibrous structure found in human tissue. (Right) Polymer nanofiber scaffolds.
Background
A 2015 Congressional Research Service Report found that 52,351 U.S. military members and civilians were non-lethally wounded in action between October 2001 and July 2015, during Operation Iraqi Freedom, Operation New Dawn, and Operation Enduring Freedom [6]. The injuries that military personnel face can be life-changing, and the DoD and other institutions are looking to tissue engineering as a means to repair these injuries. Advances in tissue engineering and regenerative medicine can potentially reduce surgical complications, speed healing time, and provide better patient outcomes for wounded soldiers.
The DoD is actively creating solutions for injuries sustained by military personnel in areas such as bone regeneration, muscle function restoration, and burn and wound care. On the basic research side, the Armed Forces Institute of Regenerative Medicine (AFIRM) is a multi-institutional, interdisciplinary network funded by the DoD and working to develop advanced treatment options for severely wounded servicemen and women. AFIRM has five research programs: extremity injury treatment, craniomaxillofacial reconstruction, skin injury treatment, vascular composite tissue allotransplantation and immunomodulation, and genitourinary and lower abdominal injury treatment. The goal of AFIRM and its numerous institutions across the globe is to use tissue engineering and regenerative medicine to address cardiovascular disease, neurological conditions, or chronic diseases, and potentially repair, replace, or regenerate damaged organs and tissues. The DoD also utilizes the tissue engineering and regenerative medicine field to treat injuries sustained by military personnel. However, a survey assessing the Technology Readiness Level (TRL) of ongoing DoD projects related to tissue engineering found that nine out 10 projects are currently at a TRL of 1. It is for this reason that alternative technologies, such as synthetic nanofiber scaffolds, are being investigated and rapidly progressing through the pre-clinical and clinical stages.
The fields of tissue engineering and regenerative medicine, as applied today, are relatively new—only about twenty years old. As the times change and the needs of healthcare providers shift, so does the focus and research of regenerative medicine. From the 1900s to the 2000s there was a change of focus in regenerative medicine—from symptomatic treatment to curative treatment [7, 8]. According to Allied Market Research, the global regenerative medicine market is expected to reach $67.5 billion by 2020. High-cost pressure on healthcare providers, due to an aging population and the increasing prevalence of chronic diseases, continues to drive interest in tissue engineering and regenerative medicine despite early setbacks and lack of significant progress [2, 7-9]. While the DoD is one of the largest funders of regenerative medicine research, their focus is on limb repair and battlefield injuries. However, tissue engineering has the potential to fix both traumatic injuries and chronic diseases. According to the Centers for Disease Control and Prevention, seven of the top 10 causes of death in 2014 were in connection to chronic diseases. Several advancements continue to push the fields of regenerative medicine and tissue engineering forward. These advancements address cardiovascular disease and neurological conditions or chronic diseases; repairing, replacing, or regenerating damaged organs and tissues; and the limitations of suitable organs for transplantation [10].
How does regenerative medicine work? The repair principles of regenerative medicine are rejuvenation, regeneration, and replacement [7, 9]. The process begins with building a scaffold that allows the body to repair itself, just like the scaffold around a building allows the workers to repair the structure. Once a functional scaffold is created, cells can be added and tissue will develop with the scaffold if the environment is right. Tissue engineering has three main components: cells, scaffolds, and biochemical signaling [1, 11, 12]. In this approach, cells are seeded on a polymeric scaffold that is then transplanted into damaged areas to repair them. With this traditional tissue engineering method, scaffolds act not only as a structural support system, but also as a conductor for cell growth. Due to the significance of the scaffold, it is imperative that the scaffold can mimic the functionality and complexity of natural tissue (Figure 3). Research is underway for other approaches to tissue engineering such as a modular approach, which involves the assembly of small cell-laden modules that are combined to form larger structures. Another approach is the idea of a scaffold- free approach. This approach consists of growing cells in the lab and getting them to self-assemble into tissues [13, 14].
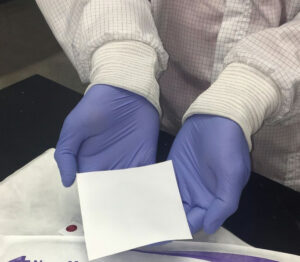
Figure 2: Phoenix Advanced Wound Care dressing.
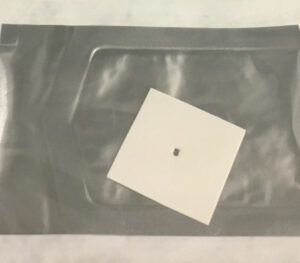
Figure 3: Atreon Orthopedics’ rotator cuff repair patch.
Current Approaches
Current medical products on the market utilize decellurized animal and human tissue. Decellularization is the process of removing cellular and immunogenic materials from tissues and organs while maintaining all the other mechanical and bioactive properties of the tissue. Think of it as doing a factory reset on a cellphone. This removes personal data, but the phone is still physically intact and can be passed on to another person for use.
Humacyte is a company developing lab-grown organs with subsequent decellularization. Their work focuses on investigational human acellular vessels that have the potential to be utilized as commercial-off-the-shelf human vascular grafts. In the current process used by Humacyte, the blood vessels are formed from banked human vascular smooth muscle cells grown into blood vessels in the lab, which are then decellularized to limit the chance of immune rejection once implanted. No cells from the patient are required for this production process. However, this lab culture process can take more than eight weeks and be very costly. Data pertaining to the recently initiated clinical trial evaluating their use in angioaccess have not been reported, but the preclinical studies used to support this ongoing clinical trial do not suggest that their performance will be superior to currently available vascular grafts made from expanded polytetrafluoroethylene, more commonly known as Gore-Tex [15-17].
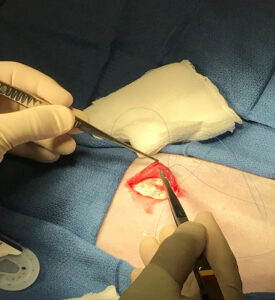
Figure 4. The Tarian Medical hernia mesh being implanted into a rabbit.
Another cell-based approach to making a vascular graft is being pursued by Cytograft, which grows flat sheets of cells which they then roll into a tube to form a blood vessel. This technology has yielded promising preclinical data and led to human clinical trials [15, 18-20]. Although these studies confirm the feasibility of using a tissue engineered vascular graft (TEVG) in humans, these cell sheet-based TEVGs have not outperformed current synthetic graft function in humans [21-23]. In fact, none of the data to date have demonstrated equivalent performance between the cell sheet TEVGs and currently available synthetic grafts.
LifeCell is another company in the tissue engineering and regenerative medicine market. LifeCell utilizes human cadaver tissue that is processed to remove cells while preserving the essential biological components and structure of the dermal matrix to support regeneration in a product called ALLODERM SELECT™. Allograft tissue can integrate into the body and has been used in numerous clinical applications, but it is extremely costly. A small patch of material can cost several thousands of dollars and some reconstructive procedures may use up to a dozen sheets.
ParaGen Technologies’ mission is to eliminate the major problems associated with synthetic and biological implants, while incorporating the advantages of each. The core of this technology is synthetic nanofiber scaffolds that mimic the physical structures in the body (see Figure 1) and allow cells to assimilate and remodel into native tissue. The nanofiber scaffold acts to provide tissue level structural support to cells, thus encouraging cell adhesion to the scaffolding and proliferation. These are the key factors that enable tissue regeneration and prevent scar formation.
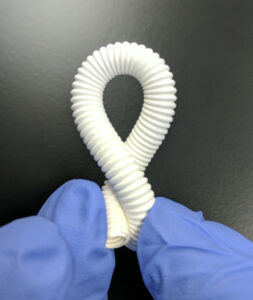
Figure 5: Vascular Genesis graft used for vascular access in dialysis patients.
ParaGen Technologies utilizes commercial- off-the-shelf polymers (i.e., the same polymers used in resorbable sutures) to create cost-effective nanofibers. Polymers are processed into fibers using electrospinning— a relatively common manufacturing process. The novel scaffold technology allows for a controlled degradation profile, a tailored fiber diameter, a specific pore size, and mechanical properties that match the native tissue. The materials are designed based on the clinical application and the devices are carefully tailored to address clinical needs for each indication.
RenovoDerm is developing a portfolio of wound care devices that increase the speed of healing for chronic wounds, while decreasing scar tissue. RenovoDerm’s first product, the Phoenix Advanced Wound Care dressing (see Figure 2) received Food and Drug Administration (FDA) approval and is currently being used in wound care clinics. Atreon Orthopedics (see Figure 3) has developed a scaffold that improves the rate of healing and overall strength of repair in rotator cuff injuries. This was tested in a sheep study and is currently in the process of being submitted to the FDA. Tarian Medical (see Figure 4) is developing a scaffold product for stronger hernia repairs with less scarring and adhesions which is being tested in rabbits. Lastly, Vascular Genesis (see Figure 5) is developing synthetic vascular grafts that remodel into healthy vascular tissue with a focus on vascular access grafts for patients undergoing hemodialysis. These vascular grafts are being tested in sheep, with the data being presented to the FDA for an interactive review and consideration as a “breakthrough device” which will significantly speed up the process for regulatory review.
Conclusion
The field of tissue engineering is progressing and moving into the clinic, which provides benefits to the DoD and civilians alike. There are products currently available on the market that fall into the category of tissue engineering such as allografts and xenografts, but lab-grown organs are still over a decade away from reaching the clinic. Additionally, there are challenges for the DoD to utilize some of these allograft materials due to the need for cold storage and short shelf life.
However, there is a new class of synthetic nanofiber scaffolds that are being used today for advanced wound care (i.e., the Phoenix Advanced Wound Care Dressing from RenovoDerm) and a suite of products for rotator cuff repair, hernia repair, and vascular graft replacement following on its heels. These synthetic nanofiber grafts are stored in ambient conditions with long shelf lives, making them great candidates for remote battlefield use (i.e., medical kits in the field) and stored at hospital centers for definitive treatment of injured military personnel and civilians. The same “plastic” that has been used for decades in resorbable sutures is now being used to re-grow organs through a process called tissue engineering. The wait for organ transplants is over, and tissue engineering is here for a better tomorrow.
References
1. Caddeo, S., Boffito, M., & Sartori, S. (2017). Tissue engineering approaches in the design of healthy and pathological in vitro tissue models. Frontiers in Bioengineering and Biotechnology, 5.
2. Tsukamoto, A., Abbot, S. E., Kadyk, L. C., Dewitt, N. D., Schaffer, D. V., Wertheim, J. A., . . . Werner, M. J. (2015). Challenging Regeneration to transform medicine. Stem Cells Translational Medicine, 5(1), 1-7.
3. Murphy, S. V., & Atala, A. (2015). New Government Accountability Office report on regenerative medicine provides an excellent assessment of the field. Stem Cells Translational Medicine, 4(12), 1371-1372.
4. Stratistics MRC. (2017, November). Tissue engineering – Global market outlook (2017-2023). Retrieved from http://www. strategymrc.com/report/tissue-engineering-market-2017
5. Sampogna, G., Guraya, S. Y., & Forgione, A. (2015). Regenerative medicine: Historical roots and potential strategies in modern medicine. Journal of Microscopy and Ultrastructure, 3(3), 101-107.
6. Fischer, H. (2015, August 7). A guide to U.S. military casualty statistics: Operation Freedom’s Sentinel, Operation Inherent Resolve, Operation New Dawn, Operation Iraqi Freedom, and Operation Enduring Freedom (Rep. No. RS22452). Retrieved https://fas.org/sgp/crs/natsec/RS22452.pdf 7. Jessop, Z. M., Al-Sabah, A., Francis, W. R., & Whitaker, I. S. (2016). Transforming healthcare through regenerative medicine. BMC Medicine, 14(1).
8. Rijt, S. V., & Habibovic, P. (2017). Enhancing regenerative approaches with nanoparticles. Journal of The Royal Society Interface, 14(129).
9. Nelson, T. J., Behfar, A., & Terzic, A. (2008). Strategies for therapeutic repair: The “R3” Regenerative Medicine Paradigm. Clinical and Translational Science, 1(2), 168-171.
10. Centers for Disease Control and Prevention. (n.d.). Chronic disease overview. Retrieved from https://www.cdc.gov/chronicdisease/ overview/index.htm
11. Forgione, A., Colombo, F., Sampogna, G., Cocozza, G., & Guraya, S. (2017). Regenerative medicine: Clinical applications and future perspectives. Journal of Microscopy and Ultrastructure, 5(1).
12. Miyachi, H., Shoji, T., Sugiura, T., Miyamoto, S., Breuer, K. C., & Shinoka, T. (2016). Clinical status of tissue engineering and regenerative medicine in cardiovascular disease. Clinics in Surgery, 1. Retrieved from http:// www.clinicsinsurgery.com/full-text/cis-v1-id1021.php
13. Duraine, G. D., Brown, W. E., Hu, J. C., & Athanasiou, K. A. (2014). Emergence of scaffold-free approaches for tissue engineering musculoskeletal cartilages. Annals of Biomedical Engineering, 43(3), 543-554.
14. Tiruvannamalai-Annamalai, R., Armant, D. R., & Matthew, H. W. (2014). A glycosaminoglycan based, modular tissue scaffold system for rapid assembly of perfusable, high cell density, engineered tissues. PLoS ONE, 9(1).
15. Dahl, S. L., Kypson, A. P., Lawson, J. H., Blum, J. L., Strader, J. T., Li, Y., . . . Niklason, L. E. (2011). Readily available tissue-engineered vascular grafts. Science Translational Medicine, 3(68).
16. Quint, C., Arief, M., Muto, A., Dardik, A., & Niklason, L. E. (2012). Allogeneic human tissue-engineered blood vessel. Journal of Vascular Surgery, 55(3), 790-798.
17. Quint, C., Kondo, Y., Manson, R. J., Lawson, J. H., Dardik, A., & Niklason, L. E. (2011). Decellularized tissue-engineered blood vessel as an arterial conduit. Proceedings of the National Academy of Sciences, 108(22), 9214-9219.
18. L’Heureux, N., Dusserre, N., Konig, G., Victor, B., Keire, P., Wight, T. N., . . . Mcallister, T. N. (2006). Human tissue-engineered blood vessels for adult arterial revascularization. Nature Medicine, 12(3), 361-365.
19. L’Heureux, N., Pâquet, S., Labbé, R., Germain, L., & Auger, F. A. (1998). A completely biological tissue-engineered human blood vessel. The FASEB Journal, 12(1), 47-56.
20. Niklason, L. E., Gao, J., Abbott, W. M., Hirschi, K. K., Houser, S., Marini, R., & Langer, R. (1999). Functional arteries grown in vitro. Science, 284(5413), 489-493.
21. Huber, T. S., Carter, J. W., Carter, R. L., & Seeger, J. M. (2003). Patency of autogenous and polytetrafluoroethylene upper extremity arteriovenous hemodialysis accesses: A systematic review. Journal of Vascular Surgery, 38(5), 1005-1011.
22. L’Heureux, N., McAllister, T. N., & De la Fuente, L. M. (2007). Tissue-engineered blood vessel for adult arterial revascularization. New England Journal of Medicine, 357(14), 1451-1453. doi:10.1056/ nejmc071536
23. Mcallister, T. N., Maruszewski, M., Garrido, S. A., Wystrychowski, W., Dusserre, N., Marini, A., . . . Lheureux, N. (2009). Effectiveness of haemodialysis access with an autologous tissue-engineered vascular graft: A multicentre cohort study. The Lancet, 373(9673), 1440-1446. doi:10.1016/ s0140-6736(09)60248-8