The exposure of military personnel to blast trauma—such as that from improvised explosive devices—can lead to ear injury and hearing deficits like sensorineural hearing loss (SNHL) and tinnitus [1]. A study conducted in 2003 found that 69 percent of returning warfighters demonstrated some level of noise-induced hearing loss (NIHL), with a majority suffering from either SNHL (29 percent) or tinnitus (30 percent) [2]. Furthermore, a 2007 study concluded that 58 percent of military personnel who suffered blast-related injuries also presented some type of hearing loss, with SNHL affecting 47 percent of those subjects [3].
Besides affecting a warfighter’s ability to function, SNHL produces long-term disabilities that require long-term management and treatment. Hearing loss (due to all causes) affects over 30 million Americans [4]. Currently, further research is necessary to define the optimum acute management and to increase the number of treatments available for hearing deficits that may occur as a result of conditions on the battlefield [5]. While hearing aids can help to some degree, they are expensive, can be uncomfortable, and do not improve speech clarity. Patients with SNHL have reduced quality of life [6], often because it causes debilitating tinnitus. Therefore, it is imperative to understand the mechanism of injury and to develop therapies based on a molecular understanding of
the changes that happen as a result of blast or noise trauma.
A direct means of assessing both inner ear morphology and function is critical for developing treatments for extremely common, yet currently untreatable, battlefield injuries such as blast- and noise-induced sensorineural hearing loss. We are focused on developing optical coherence tomography (OCT) for diagnosing inner ear disease. Using this technique, we show that osmotic disruption of the ear is visible with OCT imaging and the damage can be partially prevented by reversing the imbalance. We find OCT to be an invaluable research tool to understand and develop treatments for blast trauma to the inner ear in animal models. It can also potentially be further developed to help diagnose and treat human hearing loss.
Optical Coherence Tomography for Live Cochlea Visualization
Our ability to design better interventions for patients is frustrated by the inability to visualize the pathologic tissue damage responsible for the problem within the inner ear. It follows that an optical technique that allows non-invasive visualization of cochlear structures is necessary. OCT is a non-invasive method of visualizing soft-tissue structures on a microscale that is conceptually similar to ultrasound, but uses light rather than ultrasonic waves. OCT is a real-time imaging modality that produces high-resolution 3D images [7, 8]. By directing a light beam onto tissue and measuring backscattered light as a function of depth, OCT provides non-invasive subsurface imaging with no contact needed between the probe and tissue. OCT is FDA-approved for clinical use in imaging the eye and the coronary arteries. The inner ear is another obvious use for this optical imaging technique because the volume of tissue responsible for hearing loss and balance disorders is very small, it is surrounded by clear fluid, and it is easily damaged by invasive methodologies.
We have adapted this modern optical technique not only to visualize inner ear structures like the auditory portion, the cochlea, but also to measure vibrations of the inner ear structures using a technique called vibrometry that determines how well the cochlea is functioning (see Figure 1). Images taken with OCT clearly show the three compartments of the cochlea (scala vestibuli [SV], scala media [SM] and scala tympani [ST]), Reissner’s membrane (RM) that separates SV from SM, along with the gross anatomical structure of the Organ of Corti (tectorial membrane [TM], reticular lamina and basilar membrane [BM]), where the primary receptors for sound—the hair cells— reside (see Figure 1B). Additionally, vibrometry of different Organ of Corti structures (e.g., BM and TM) show the sharp tuning and large gains of cochlear amplification (see Figure 1C and D; BM: 62.3±0.6 dB, TM: 65.3±0.5 dB, n=3, 11 kHz). Using OCT, we can now diagnose changes that happen in the cochlea in response to blast injury or noise-exposure.
Blast- and Noise-induced Models of Hearing Loss
The cochlea is the body’s most sensitive pressure transducer because it houses the hair cells, which use their receptor organelle, the hair bundle, to transduce mechanical energy from pressure waves into electrochemical signals. There are two types of hair cells: inner hair cells (IHCs) and outer hair cells (OHCs). IHCs send sensory information to the brain, while OHCs amplify and tune the response of the ear. OHCs are highly mechanosensitive and easily damaged by loud sounds that result in strong pressure waves in the ear. Therefore, the classical explanation for hearing loss due to blast or noise exposure is trauma to the hair cells. Hair cell death occurs through a variety of pathways [9, 10] that result in permanent hearing loss, which can be measured as elevated auditory thresholds.
However, an additional mechanism of noise induced hearing loss, known as cochlear synaptopathy, also occurs. Synaptopathy results in reduced communication between the hair cell and its afferent nerve fiber either via a decrease in the number of communication sites, known as synapses, or a degeneration of the postsynaptic auditory nerve fiber. The mechanism of synaptopathy is thought to be primarily due to an excess release of the hair cell afferent neurotransmitter, glutamate, after hair cell overstimulation, or secondarily to extracellular adenosine 5′-triphosphate (ATP) release from other traumatized cells, which leads to Ca2+ channel activation [11, 12]. This results in the toxic entry of ions and water into synaptic boutons [13–15], which damages the dendrite and produces loss of synaptic ribbons, the presynaptic components of hair cells [16–18]. Loss of synapses and auditory neurons does not necessarily elevate the threshold of hearing, but instead affects neural encoding at higher sound intensities, so it has been called “hidden” hearing loss [19]. There are no effective medical treatments to prevent hearing loss via either mechanism after traumatic noise exposure.
As with noise, inner ear damage from improvised explosive devices, which causes a primary blast injury, results from the direct effect of the high pressure wave upon the tissue [20]. To determine if the etiology of blast-wave exposure matched that of noise exposure, we custom-built a blast chamber to deliver blast waves to mice (see Figure 2A–D). This system is pressurized with compressed air, which when released, produces a single compression wave that travels down a metal tube and develops a shock front that creates a blast wave by the time it reaches the mouse. Our chamber could generate peak pressures of up to 186 kPa, corresponding to a sound intensity of 199 dB SPL (sound pressure level) at the position of the mouse [21].
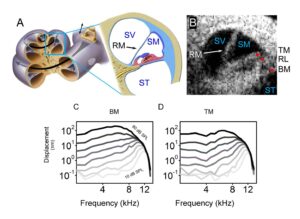
Figure 1. Optical coherence tomography and vibrometry in the mouse cochlea. (A) Illustration of a cross-sectional view of the cochlea, with expanded view of the apical turn where this data was collected. SV, scala vestibuli; SM, scala media; ST, scala tympani; RM, Reissner’s membrane. (B) Cross-sectional OCT image of the mouse cochlea. Red dots highlight common locations for vibrometry measurements. TM, tectorial membrane; RL, reticular lamina; BM, basilar membrane. Vibratory responses from (C) BM and (D) TM in the live mouse cochlea shown in (B) display tuned responses centered near the BF (best frequency ~12 kHz). Adapted from [22].
OCT Can Diagnose Dynamic Changes in the Inner Ear
Equipped with OCT as a diagnostic tool, a 2018 study showed that dynamic inner ear changes due to blast trauma could be elucidated [22]. Serially imaging the cochlea of anesthetized mice exposed to a single blast wave approximating that of a roadside bomb (peak pressures 130±9 kPa [~196 dB SPL], see Figure 2E) showed that during the first three hours after the blast, there was progressive bulging of Reissner’s membrane—consistent with an increase in endolymph volume, or endolymphatic hydrops [23] (see Figure 3A). By one day after the blast, Reissner’s membrane returned to a normal position where it remained for at least one week (see Figure 3B–D). In contrast, unexposed control mice demonstrated no change in Reissner’s membrane (see Figure 3E), indicating that OCT imaging does not cause endolymphatic hydrops. Following euthanasia, Reissner’s membrane progressively shifted inward (see Figure 3F), consistent with reduced endolymph secretion by the stria vascularis post-mortem [24, 25]. Quantifying endolymph volume within scala media confirmed OCT is able to assess dynamic endolymphatic fluid volume changes (see Figure 3G). Furthermore, because the presence of endolymphatic hydrops is indicative of a change in osmotic balance of the cochlea, we looked for further damage in the ear that might cause, or result from, this imbalance.
After visualizing gross morphological changes in live mice after blast trauma, Kim et al. [22] used in vitro microscopy techniques with finer resolution to visualize cells and their different structures. High-resolution, scanning electron microscopy revealed immediate hair bundle damage in the cochlear base (see Figure 4A– B) that was not present in unexposed control animals. Labeling hair cells in the cochlea with fluorescent markers showed bundle damage was followed by outer hair cell loss one week post blast exposure (see Figure 4C–D). In contrast to the localized loss of outer hair cells, ribbon synapses were lost throughout the cochlea seven days after the blast (see Figure 4E–H). These two different patterns of damage highlight the distinct mechanisms underlying hair cell death and neuronal trauma [21, 22]. The pattern of basal hair cell loss is well-established and is due to the large forces applied to the stereociliary bundles [10, 26]. Because there is no obvious explanation for why the pattern of neuronal damage did not match that of the hair cells, Kim et al. [22] hypothesized that the former was due to the endolymphatic hydrops that developed.
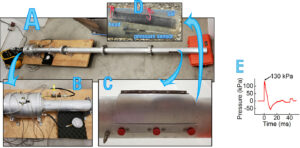
Figure 2. The blast chamber. (A) A picture of the blast chamber. (B) A zoom in of the blast chamber and pressure gauge. (C) A close up of the end of the blast tube, which houses the mouse and (D) the protective sheath and the pressure sensor. (E) The blast wave pressure monitored by a sensor positioned just below the mouse.
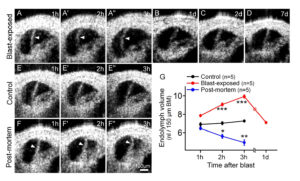
Figure 3. Blast exposure produces transient endolymphatic hydrops. (A) Endolymphatic
hydrops progressively developed within the first three hours after blast exposure. Images are from one representative mouse. (B–D) OCT images from different representative mice showed normal endolymph volume one, two and seven days after blast exposure. (E) OCT
images from a representative live control mouse over three hours demonstrated normal endolymph volume. (F) OCT images from an unexposed control mouse followed for three hours after euthanasia demonstrated progressive reductions of endolymph volume. (G) Scala
media volume over time in blast-exposed mice, living control mice, and unexposed control mice post-mortem. *P<0.05, **P<0.01, ***P<0.001. Adapted from [22].
Osmotic Imbalance Leads to Cochlear Synaptopathy
To test if endolymphatic hydrops caused synaptopathy throughout the cochlea, Kim et al. [22] applied the simple chemical principles of osmosis and diffusion. Application of varying tonicity solutions to the middle ear draws fluids into or out of the perilymphatic and endolymphatic spaces within the fluid-filled cochlea (see Figure 5A). Such fluid application is a safe clinical technique [27–29]. A solution with fewer solutes than that found in perilymph and endolymph (hypotonic) would force water into the cochlea, which should result in endolymphatic hydrops. In contrast, a hypertonic solution, i.e., a solution that has a higher solute concentration than perilymph and endolymph, should drain fluids from the inner ear via osmosis. This is what is needed to reverse endolymphatic hydrops. Solutions with a similar salt concentration as body fluids, normotonic solutions, should not change the endolymph volume.
Applying a hypotonic solution for 5.5 hours did indeed induce endolymphatic hydrops in mice (see Figure 5B). Furthermore, measuring cochlear function using the vibrometry aspect of OCT showed that these mice had normal vibratory responses (see Figure 5C–D). Therefore, isolated endolymphatic hydrops (i.e., not associated with mechanical trauma of stereociliary bundles) does not alter the OHC-based mechanical response. However, synaptic ribbon counts were reduced after the hypotonic challenge to a level similar to that found after blast exposure even though there was no loss of OHCs (n=4) (see Figure 5E–G). Thus, endolymphatic hydrops in isolation causes cochlear synaptopathy.
The Mechanism of Synaptopathy After Trauma is Similar in the Ear and Brain
Excitotoxicity—the toxic effects of too much neurotransmitter release due to overstimulation—is a known mechanism for synaptopathy,not only in the cochlea [13–16], but also in the central nervous system after traumatic brain injury (TBI) [30] and likely blast-induced neurotrauma seen in military personnel [31]. Kim et al. [22] tested if this was the mechanism underlying hydrops-induced synaptopathy in the cochlea. For these studies, a more direct method (perilymphatic perfusion of either normotonic or hypotonic artificial perilymph over one hour) was used to cause endolymphatic hydrops and study both the pre- (using CtBP2 immunolabeling) and post-synaptic (using Homer immunolabeling [32]) sides of the IHC synapse (see Figure 6). Mice perfused with hypotonic perilymph again developed hydrops and had reduced CtBP2 and Homer puncta counts that were less well co-localized. In these mice, the CtBP2 and Homer puncta counts and co- localization could be preserved by mitigating the effects of the released neurotransmitters by blocking the postsynaptic AMPA glutamate receptor with the drug, CNQX (see Figure 6E). Thus, blocking hydrops-induced excitotoxicity is a viable means of protecting synapses. However, because blocking synaptic transmission can have deleterious side effects [33], we searched for a simpler therapeutic.
Osmotic Stabilization as a Treatment for Blast Injury
By causing endolymphatic hydrops with a hypotonic solution, Kim et al. [22] hypothesized that endolymphatic hydrops could be reversed using a hypertonic solution applied to the middle ear. Furthermore, because synaptopathy resulted from hydrops, neutralizing the osmotic imbalance should prevent it. To test if endolymphatic hydrops could be reversed, OCT images were taken three hours after blast-exposure when endolymphatic hydrops had developed. Addition of hypertonic, artificial perilymph to the middle ear did reverse endolymphatic hydrops, but normotonic saline did not (see Figure 7A– C). Next, OHCs and synapses were assessed to see if hypertonic saline could protect from outer hair cell loss and synaptopathy. After blast exposure, the hypertonic solution was applied to the round window, and two months later, hair cells and synapses were counted throughout the cochlea (see Figure 7D–F).
While unexposed control mice had no OHC loss, blast-exposed mice that were not treated with the hypertonic solution had substantial OHC loss in the cochlear base, scattered loss in the middle, and no loss in the apex. Hair cell counts were the same in these untreated ears and those treated with normotonic or hypertonic saline (see Figure 7G). There were substantial reductions in the number of synaptic ribbons per OHC and IHC in untreated ears and in ears treated with normotonic artificial perilymph (see Figure 7H–I). However, treatment with the hypertonic solution prevented most of this synaptic loss. Thus, hypertonic treatment of post-traumatic endolymphatic hydrops preserved roughly half the synaptic ribbons that would have been lost without treatment, but had no impact on OHC loss.
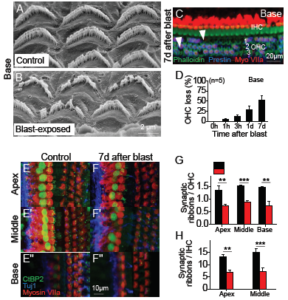
Figure 4. Blast exposure causes stereociliary trauma, hair cell loss, and cochlear synaptopathy. (A, B) Scanning electron microscopic images of OHC stereociliary bundles in representative control and blast-exposed mice. (C) The cochlear epithelium seven days after blast exposure. Immunolabeling was done to visualize all hair cells (Myosin VIIa) and outer hair cells (OHCs) (Prestin). Representative locations of missing hair cells are shown (arrowheads). (D) Quantification of OHC loss seven days after blast exposure in the basal regions. (E, F) The cochlear epithelium seven days after blast exposure. Immunolabeling was done to visualize synaptic ribbons in hair cells (CtBP2), hair cells (Myosin VIIa), and auditory neurons (Tuj1). (G, H) Quantification of synaptic ribbons per OHC and IHC in control and blast-exposed mice. **P<0.01, ***P<0.001. Adapted from [22].
Discussion
The data presented in Kim et al. [22] reveal several fundamental mechanisms that underlie blast-induced sensorineural hearing loss (see Figure 8). The most significant finding of Kim et al. [22] is that osmotically stabilizing the cochlear fluids after the blast exposure prevents cochlear synaptopathy, even though hair cell fate remains unchanged [22, 34]. The initiating step of the problem is the mechanically induced trauma to the OHC stereociliary bundle. The fate of these traumatized OHCs is set immediately and is not changed by any of the tested interventions. Clearly, there is a need for further research to understand how to preserve hair cells after traumatic events. As it is now feasible to integrate high-throughput expression analysis data from independent experiments [35], perhaps studying expression differences when comparing hair cells that survive after bundle damage [36] against those hair cells that succumb to bundle damage [22] could elucidate the molecular causes of hair cell degeneration.
In contrast to the fate of the hair cells, cochlear synaptopathy has a delayed-onset involving several steps. First, endolymphatic hydrops occurs, likely because K+ is not removed fast enough from the endolymph after stereociliary trauma. We showed that this, like brain edema after TBI, can be treated by osmotic stabilization— protecting from further symptoms that may occur downstream or concomitantly [22, 37]. Next, hair cells release excess neurotransmitters, which may occur because the post-traumatic endolymphatic hydrops over-stimulates them or because nearby damaged cells release ATP that creates calcium waves across the epithelium [12, 38]. The excitotoxicity of excess glutamate causes ion and H2O entry into synaptic boutons, and this swelling is associated with either temporary or permanent damage [13–15]. Finally, the loss of the bouton leads to loss of synaptic ribbons inside the hair cell [16]. Because synapse loss was reduced with a blocker of excitotoxicity, this suggests that antiexcitotoxic therapies like those used for TBI [37] may also prevent cochlear synaptopathy after blast injury.
The results of our study also suggest a novel treatment for blast-induced trauma that will protect warfighters from hidden hearing loss, for which there is currently no reliable and validated diagnostic test [39]. Osmotically stabilizing the inner ear after noise exposure may offer an important therapeutic approach to preserve hearing. We show that treatment is efficacious in mice using a middle ear injection technique that is commonly used in the clinic and could be used on the battlefield as it only requires a concentrated salt solution in an ear drop. Similarly, such injections may be an alternative treatment for vestibular diseases, like Meniere’s disease, in which endolymphatic hydrops causes episodic vertigo, fluctuating hearing loss, and roaring tinnitus [23, 40–43], negatively impacting a patient’s quality of life. Future work may seek to reveal the exact molecular mechanism underlying the permanent cochlear synaptopathy. To this end, noise-exposure models that we showed also develop endolymphatic hydrops can be used to understand if both modalities of inner ear trauma have similar etiologies [22] and to create more targeted interventions that allow hearing preservation in stressful environments.
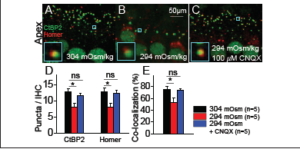
Figure 6. Excitotoxic block prevents cochlear synaptopathy induced by hydrops. (A–C) The cochlear epithelium harvested from representative mice perfused for 1 hour with 304 mOsm (A), 294 mOsm (B), or 294 mOsm + 100 μM CNQX (C) artificial perilymph. Immunolabeling was done to visualize pre-synaptic ribbons in IHCs (CtBP2) and post-synaptic auditory nerve boutons (Homer). Co-localization of the pre- and post-synaptic terminals is shown (insets). (D) Hypotonic challenge reduced CtBP2 and Homer counts. Blocking glutamate with CNQX preserved both the pre- and post-synaptic terminals. *P<0.05; ns, not significant. (E) Hypotonic challenge reduced the rate of CtBP2 and Homer co-localization; this effect was also reversed with CNQX. Adapted from [22].
However, the outcomes vary greatly. While the variation is not entirely understood, an accepted contributing factor is the choice of the length of the prostheses [44, 45]. However, there is currently no imaging technology that can provide pre-operative or intra-operative quantitative guidance on optimal prosthetic length. Scar tissue formation around the prosthesis can also adversely affect hearing outcomes, and there is no reliable way to identify this problem. Therefore, if the outcome is poor it is often difficult to determine the cause and whether a revision surgery is indicated [46].
Because OCT has both the spatial and temporal resolution to measure the morphological features and function of the ear, it may provide this much needed diagnostic ability in humans, leading to better outcomes and lower health care costs. The challenge with utilizing OCT for interrogating the middle ear space is access, which requires adaptations in the current hardware so that entry can be gained through the ear canal or Eustachian tube. Thus, continuing technological advancements on the OCT system can lead to better diagnostics that can swiftly impact and improve clinical care of warfighters after blast injury.
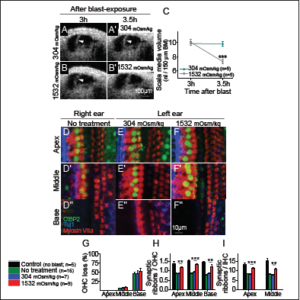
Figure 7. Osmotic treatment of endolymphatic hydrops partially rescues synaptic ribbon loss after blast exposure. (A, B) Representative mice were treated with normotonic or hypertonic challenge three hours after blast exposure. Repeat images were taken 30 minutes later. (C) Normotonic artificial perilymph had no impact on post-traumatic endolymphatic hydrops whereas hypertonic artificial perilymph normalized endolymphatic volume. (D–F) The cochlear epithelium from representative mice two months after blast exposure. Mice had either no treatment (D), normotonic, artificial perilymph application to the middle ear after the blast (E), or hypertonic, artificial perilymph application to the middle ear after the blast (F). Immunolabeling was done to visualize synaptic ribbons in hair cells (CtBP2), hair cells (Myosin
VIIa), and auditory neurons (Tuj1). (G–I) Quantification of OHC loss and synaptic ribbons per OHC and IHC. Hypertonic artificial perilymph reduced the loss of synaptic ribbons but did not affect the degree of OHC loss. **P<0.01, ***P<0.001. Adapted from [22].
References
1. Dougherty, A. L., MacGregor, A. J., Han, P. P., Viirre, E., Heltemes, K. J., & Galarneau, M. R. (2013). Blast-related ear injuries among U.S. military personnel. Journal of Rehabilitation Research & Development, 50(6), 893–904. doi:10.1682/ JRRD.2012.02.0024
2. Helfer, T. M., Jordan, N. N., & Lee, R. B. (2005). Post deployment hearing loss in U.S. Army soldiers seen at audiology clinics from April 1, 2003, through March 31, 2004. American Journal of Audiology, 14(2), 161–168. doi:10.1044/1059-0889(2005/018)
3. Cave, K. M., Cornish, E. M., & Chandler, D.W. (2007). Blast injury of the ear: clinical update from the global war on terror. Military Medicine, 172(7), 726–730. doi:10.7205/MILMED.172.7.726
4. Pleis, J. R., & Lethbridge-Cejku, M. (2006).Summary health statistics for U.S. adults: National Health Interview Survey, 2005. Vital Health Statistics 10(232), 1–153.
5. Muzaffar, S. J., Orr, L., Rickard, R. F., Coulson, C. J., & Irving, R. M. (2018). Mitigating noise-induced hearing loss after blast injury. Trauma, 1–7.doi:10.1177/146040861875519
6. Yankaskas, K. (2013). Prelude: noise-induced tinnitus and hearing loss in the military. Hearing Research, 295, 3–8.doi:10.1016/j.heares.2012.04.016
7. Huang, D., Swanson, E. A., Lin, C. P., Schuman, J. S., Stinson, W. G., Chang, W., . . . Puliafito, C. A., et al (1991). Optical coherence tomography. Science, 254(5035),1178–1181. doi:10.1126/science.1957169
8. Izatt, J. A., Kulkarni, M. D., Wang, H. W., Kobayashi, K., & Sivak, M. V. (1996). Optical coherence tomography and microscopy in gastrointestinal tissues. IEEE Journal of Selected Topics in Quantum Electronics, 2(4), 1017–1028. doi:10.1109/2944.577331
9. Yang, C. H., Schrepfer, T., & Schacht, J. (2015). Age-related hearing impairment and the triad of acquired hearing loss. Frontiers in Cellular Neuroscience, 9(276), 1–12. doi:10.3389/fncel.2015.00276
10. Furness, D. N. (2015). Molecular basis of hair cell loss. Cell and Tissue Research, 361(1), 387–399. doi:10.1007/s00441-015-2113-z
11. Mammano, F., Frolenkov, G. I., Lagostena, L., Belyantseva, I. A., Kurc, M., Dodane, V., . . . Kachar, B. (1999). ATP-Induced Ca(2+) release in cochlear outer hair cells: localization of an inositol triphosphate-gated Ca(2+) store to the base of the sensory hair bundle. Journal of Neuroscience,19(16), 6918–6929. doi:10.1523/JNEUROSCI. 19-16-06918.1999
12. Piazza, V., Ciubotaru, C. D., Gale, J. E., & Mammano, F. (2007). Purinergic signalling and intercellular Ca2+ wave propagation in the organ of Corti. Cell Calcium, 41(1), 77–86. doi:10.1016/j.ceca.2006.05.0011.
13. Pujol, R., & Puel, J. L. (1999). Excitotoxicity, synaptic repair, and functional recovery in the mammalian cochlea: a review of recent findings. Annals of the New York Academy of Sciences, 884, 249–254. doi:10.1111/j.1749-6632.1999.tb08646.x
14. Mayer, M. L., & Westbrook, G. L. (1987).Cellular Mechanisms Underlying Excitotoxicity. Trends in Neurosciences, 10(2), 59–61. doi:10.1016/0166-2236(87)90023-3
15. Choi, D. W., & Rothman, S. M. (1990). The role of glutamate neurotoxicity in hypoxic- ischemic neuronal death. Annual Review of Neuroscience, 13, 171–182. doi:10.1146/ annurev.ne.13.030190.001131
16. Moser, T., & Starr, A. (2016). Auditory neuropathy– neural and synaptic mechanisms. Nature Reviews Neurology, 12(3), 135–149. doi:10.1038/nrneurol.2016.10
17. Lin, H. W., Furman, A. C., Kujawa, S. G., & Liberman, M. C. (2011). Primary neural degeneration in the Guinea pig cochlea after reversible noise-induced threshold shift. Journal of the Association for Research in Otolaryngology, 12(5), 605–616.doi:10.1007/s10162-011-0277-0
18. Kujawa, S. G., & Liberman, M. C. (2009). Adding insult to injury: cochlear nerve degeneration after “temporary” noise-induced hearing loss. Journal of Neuroscience, 29(45), 14077–14085. doi:10.1523/JNEUROSCI. 2845-09.2009
19. Schaette, R., & McAlpine, D. (2011). Tinnitus with a normal audiogram: physiological evidence for hidden hearing loss and computational model. Journal of Neuroscience, 31(38), 13452–13457. doi:10.1523/JNEUROSCI.2156-11.2011
20. Garner, J., & Brett, S. J. (2007). Mechanisms of injury by explosive devices. Anesthesiology Clinics, 25(1), 147–160, x. doi:10.1016/j.anclin.2006.11.002
21. Cho, S. I., Gao, S. S., Xia, A., Wang, R., Salles, F. T., Raphael, P. D., . . . Oghalai, J. S. (2013). Mechanisms of hearing loss after blast injury to the ear. PLoS One, 8(7), e67618. doi:10.1371/journal.pone.0067618
22. Kim, J., Xia, A., Grillet, N., Applegate, B. E., & Oghalai, J. S. (2018). Osmotic stabilization prevents cochlear synaptopathy after blast trauma. Proceedings of the National Academy of Sciences, 115(21), E4853– E4860. doi:10.1073/pnas.1720121115
23. Schuknecht, H. F. (1993) Pathology of the ear, 2nd Ed. Malvern, PA: Lea & Febiger.
24. Zdebik, A. A., Wangemann, P., & Jentsch, T. J. (2009). Potassium ion movement in the inner ear: insights from genetic disease and mouse models. Physiology (Bethesda), 24, 307–316. doi:10.1152/physiol.00018.2009
25. Patuzzi, R. (2011). Ion flow in stria vascularis and the production and regulation of cochlear endolymph and the endolymphatic potential. Hearing Research, 277(1-2), 4–19. doi:10.1016/j.heares.2011.01.010
26. Liu, C. C., Gao, S. S., Yuan, T., Steele, C., Puria, S., & Oghalai, J. S. (2011). Biophysical mechanisms underlying outer hair cell loss associated with a shortened tectorial membrane. Journal of the Association for Research in Otolaryngology, 12(5), 577– 594. doi:10.1007/s10162-011-0269-0
27. Oghalai, J. S., Tonini, R., Rasmus, J., Emery, C., Manolidis, S., Vrabec, J. T., & Haymond, J. (2009). Intra-operative monitoring of cochlear function during cochlear implantation. Cochlear Implants International, 10(1), 1–18. doi:10.1002/cii.372
28. Cooper, N. P., & Rhode, W. S. (1996). Fast travelling waves, slow travelling waves and their interactions in experimental studies of apical cochlear mechanics. Auditory Neuroscience, 2(3), 207–217.
29. Wenzel, G. I., Xia, A., Funk, E., Evans, M. B., Palmer, D. J., Ng, P., . . . Oghalai, J. S. (2007). Helper-dependent adenovirus-mediated gene transfer into the adult mouse cochlea. Otology & Neurotology, 28(8), 1100–1108.
30. Margulies, S., Hicks, R., et al. (2009). Combination therapies for traumatic brain injury: prospective considerations. Journal of Neurotrauma, 26(6), 925–939. doi:10.1089/neu.2008-0794
31. Cernak, I., & Noble-Haeusslein, L. J. (2010). Traumatic brain injury: an overview of pathobiology with emphasis on military populations. Journal of Cerebral Blood Flow & Metabolism, 30(2), 255–266. doi:10.1038/jcbfm.2009.203
32. Martinez-Monedero, R., Liu, C., Weisz, C., Vyas, P., Fuchs, P. A., & Glowatzki, E. (2016). GluA2-containing AMPA receptors distinguish ribbon-associated from ribbon less afferent contacts on rat cochlear hair cells. eNeuro, 3(2). doi:10.1523/ENEURO. 0078-16.2016
33. Ikonomidou, C., & Turski, L. (2002). Why did NMDA receptor antagonists fail clinical trials for stroke and traumatic brain injury? Lancet Neurology, 1(6), 383-386. doi:10.1016/S1474-4422(02)00164-3
34. Wallis, C. (2018, August 1). Ba-Boom! There goes your hearing. Scientific American, 319(2), 24. doi:10.1038/scientificamerican0818-24
35. Hertzano, R., & Elkon, R. (2012). High throughput gene expression analysis of the inner ear. Hearing Research, 288(1-2), 77– 88. doi:10.1016/j.heares.2012.01.002
36. Jia, S., Yang, S., Guo, W., & He, D. Z. (2009). Fate of mammalian cochlear hair cells and stereocilia after loss of the stereocilia. Journal of Neuroscience, 29(48), 15277–15285. doi:10.1523/JNEUROSCI.3231-09.2009-
37. Kochanek, P. M., Jackson, T. C., Ferguson,N. M., Carlson, S. W., Simon, D. W., Brockman, E. C., . . . Dixon, C. E. (2015). Emerging therapies in traumatic brain injury. Seminars in Neurology, 35(1), 83–100. doi:10.1055/s-0035-1544237
38. Chan, D. K., & Rouse, S. L. (2016). Sound-induced intracellular Ca2+ dynamics in the adult hearing cochlea. PLoS One, 11(12), e0167850. doi:10.1371/journal.pone.016785037.
39. Plack, C. J., Leger, A., Prendergast, G., Kluk, K., Guest, H., & Munro, K. J. (2016). Toward a Diagnostic Test for Hidden Hearing Loss. Trends in Hearing, 20.doi:10.1177/2331216516657466
40. Sajjadi, H., & Paparella, M. M. (2008). Meniere’s disease. Lancet, 372(9636), 4 0 6 – 4 1 4 . d o i : 1 0 . 1 0 1 6 / S 0 1 4 0 -6736(08)61161-7
41. Schuknecht, H. F. (1976). Pathophysiology of endolymphatic hydrops. European Archives of Otorhinolaryngology, 212(4), 253–262.
42. Salt, A. N., & Plontke, S. K. (2010). Endolymphatic hydrops: pathophysiology and experimental models. Otolaryngologic Clinics of North America, 43(5), 971–983.doi:10.1016/j.otc.2010.05.007
43. Valk, W. L., Wit, H. P., Segenhout, J. M., Dijk, F., van der Want, J. J., & Albers, F. W. (2005). Morphology of the endolymphatic sac in the guinea pig after an acute endolymphatic hydrops. Hearing Research, 202(1-2), 180–187. doi:10.1016/j. heares.2004.10.010
44. Morris, D. P., Bance, M., van Wijhe, R. G., Kiefte, M., & Smith, R. (2004). Optimum tension for partial ossicular replacement prosthesis reconstruction in the human middle ear. Laryngoscope, 114(2), 305–308. doi:10.1097/00005537-200402000-00024
45. Ulku, C. H., Cheng, J. T., Guignard, J., & Rosowski, J. J. (2014). Comparisons of the mechanics of partial and total ossicular replacement prostheses with cartilage in a cadaveric temporal bone preparation. Acta Oto-Laryngolgica, 134(8), 776–784. doi:10.3109/00016489.2014.898187
46. Stone, J. A., Mukherji, S. K., Jewett, B. S., Carrasco, V. N., & Castillo, M. (2000). CT evaluation of prosthetic ossicular reconstruction procedures: what the otologist needs to know. Radiographics, 20(3), 593–605. doi:10.1148/radiographics. 20.3.g00ma03593